M H Ogle (1) , F M Burdekin (2) and I Hadley (3)
(1,3) TWI
(2) Formerly Dept of Civil and Structural Engineering, UMIST
Paper presented at Materials selection requirements for Civil Structures, 56th IIW Annual Assembly 6-11 July 2003, Bucharest, Romania.
Abstract
The current range of structural steel products available in Europe is reviewed, together with their material qualities and typical costs. The factors influencing choice of steel grade are discussed. The development of Charpy selection values in UK steel building and bridge codes is described and the latest rules are compared with the new European brittle fracture rules for steel structures. The criteria for the selection of through-thickness properties are discussed with reference to the new European welding and design codes.
1. Introduction
1.1 Structural steel applications
Steel continues to be one of the most important structural materials for urban construction in many counties in the world. Its speed of construction, lightweight, compactness and good appearance often give it an overall advantage when competing against its main rival reinforced or prestressed concrete. The main perceived disadvantages, fire and corrosion resistance, are today less significant factors in the equation than they used to be. This is due to development of light weight fire protection systems and the realisation that the long-term durability of concrete in exposed environments can incur similar or greater maintenance costs than in the case of steel.
A further factor in favour of steel is the greater opportunity to automate shop production, the ease of transport and the rapid site assembly of structure with full strength joints using the minimum of falsework. As the cost of labour increases in developing countries so these factors are likely to favour structural steel.
It must not be forgotten however that steel and concrete work well together in partnership and many of today's urban structures employ composite construction where structural steel for the main framework and girders and reinforced concrete for floors, core wall and deck slabs are used to advantage.
The greatest tonnage of structural steel is used in building structures. These range across the full spectrum from lightweight portal frame industrial premises to multi-storey offices, warehouses, retail outlets, heavy industrial workshops, power stations and process supporting structures. Except for the heavy end of the market, the main steel products used are rolled sections and sheeting. At the light end of the market the shop joints are usually made with fillet welds. Site joints are usually bolted. At the heavy end members may be fabricated from plate with thicknesses exceeding 100mm.
A smaller, but still very important, market for steel is for road and rail bridges. Much of this market concerns replacement of 19 th Century bridges which have come to the end of their useful life. The speed of replacement, which is often limited to a possession of a few hours, is an important factor in favour of structural steel. This sector mainly uses steel plate which is fabricated by welding into I or box girders.
1.2 Choice of product forms and material girders
The designer and architect have a wide range of steel products at their disposal. At the light to medium end of the market these may be obtained from a steel stockholder. At the heavy end they are more likely to be obtained by direct order by the fabricator from the rolling mill.
In Europe the national steel product standards have now been replaced by European equivalents. This has had the effect of sourcing material from a wider range of manufacturers, some of whom are outside Europe. In an increasingly competitive world, materials and products are more likely to be sourced on the basis of price alone. The hidden danger in this situation is that steels may be supplied from time to time which only just meet the requirements of the product standard, whereas a fabricator used to buying from more local sources may have been used to steels which were generally comfortably within the product standard requirements. This can give rise to fabrication and integrity problems, as will be discussed later.
Table 1 Commonly available range of structural steel products
Generic form | Products | Number of sizes (approx) | Thickness range, (mm) | Cross sectional dimension range, (mm) | Steel grades |
S235-S275 | S355 | S420-S460 | S690 |
Sheet |
Cladding, purlins floor troughs, etc |
Numerous |
0.9-3.6 |
Up to 1200 |
✔ |
✔ |
|
|
Plate |
|
Numerous |
4-250 |
600-4000 |
✔ |
✔ |
✔ |
✔ |
Sections |
Angles, channels, tees |
~125 |
5-35 |
25-430 |
✔ |
✔ |
|
|
|
Beams, joists |
~90 |
4-37 |
127-920 |
✔ |
✔ |
|
|
|
Columns |
30+ |
6-77 |
150-475 |
✔ |
✔ |
|
|
|
Rectangular hollow sections |
300+ |
2-16 |
20-450 |
✔ |
✔ |
|
|
|
Circular hollow sections |
Numerous |
2.5-40 |
21-508 |
✔ |
✔ |
|
|
The range of structural steel products commonly available today in the European Market is shown in Table 1. This illustrates the wide range of thicknesses and grades available to the designer. The European strength grades are now denoted by the nominal yield strength. For example S355 will have a minimum specified yield strength of 355N/mm2 for the thinnest products, the specified strength generally reducing marginally as the thickness increases. The current European structural steel product standards are shown in Table 2.
Table 2 Current European structural steel product standards
Standard | Products covered | Steel type process route | Strength grades (R e - N/mm 2 | Minimum Charpy test, temperature, °C (1) |
Plates | Sections | 235 | 275 | 355 | 420 | 460 | 500 to 960 | -30 | -40 | -50 | -60 |
EN 10025 |
✔ |
Open |
Non alloy |
✔ |
✔ |
✔ |
|
|
|
( ✔) |
|
|
|
EN 10113 (2) |
✔ |
[Open] (4) |
Weldable fine grain |
|
✔ |
✔ |
✔ |
✔ |
|
|
|
✔ |
|
EN 10155 (2) |
✔ |
Open |
Weathering |
|
|
✔ |
|
|
|
( ✔) |
|
|
|
EN 10210 |
|
Hollow |
Hot finished |
|
✔ |
✔ |
|
|
|
|
|
✔ |
|
EN 10219 |
|
Hollow |
Cold formed |
|
✔ |
✔ |
|
|
|
|
|
✔ |
|
EN 10137 (2) |
✔ |
|
AT or PH (3) |
|
|
|
|
✔ |
✔ |
|
|
|
✔ |
Notes:
1. Mostly 27 Joules minimum energy requirement. Brackets indicate that 40 Joules is required at -20°C which is approximately equivalent to 27 Joules at an effective test temperature of -30°C.
2. To be subsumed into a new edition of EN10025 to be published in 6 Parts.
3. Quench and tempered or precipitation hardened.
4. Rarely supplied owing to the lack of normalising furnace facilities in most section rolling mills.
This shows the range of products, steel types, strength and impact grades available to the designer. The main bulk of structural steelwork is made using non alloy steel to EN 10025 which offers yield strengths up to 355N/mm 2 and 27 Joule Charpy impact values down to an effective test temperature of -30°C. The fine grain steels to EN 10113, which are either normalised, normalised rolled or thermomechanically rolled, offer yield strengths up to 460N/mm 2 and impact testing down to -50°C. This latter specification is mostly used for plate rather than sections, although it covers both (see Note 4 in Table 2). Higher plate strengths still are available though EN 10137 with a marginally lower Charpy impact test temperature.
The selection of strength grades is discussed in Section 2 below and impact quality requirements in Section 3. Whilst the selection of strength grade does not pose too much difficulty for structural steel designers, the selection of impact quality is often a matter of mystery. For this reason Section 3 provides a detailed insight into the development of guidance rules on this topic.
1.3 Material quality options
The new European structural steel product standards contain a number of important choices for both the designer and the fabricator. These choices will have implications for both the structural integrity and the economy of the structures. The main options available are listed in Table 3. This list covers the main options available in one or more of the following standards: EN 10025, EN 10113 and EN 10137 (see Table 2).
Table 3 Typical options available in European Structural Steel Product Standards
Aspect | Options |
Steel making process |
Reporting of process used. Specific process required. Normalising rolling (N) required. |
Chemical control |
Method of de-oxidation specified. Individual element limits e.g. C, Cu. Carbon equivalent value limitation. Product analysis required. |
Tensile testing |
Increased frequency of testing. Alternative test piece shape. |
Impact testing |
Transverse impact values required. Non-standard test temperature. Increased frequency of testing. Verification of JR quality by test. |
Through thickness testing |
Minimum Z quality specified. |
Inspection of final product |
Increased extent of inspection. Inspection by purchaser. Type of inspection document. Surface inspection by purchaser. Ultrasonic inspection for internal flaws. |
Fabrication processes |
Suitable for cold rolling of plate and strip. Suitable for cold drawing of bars. Suitable for flanging of flat products. Suitable for splitting of heavy sections. |
Coating processes |
Suitable for hot dip galvanising or enamelling. |
The purpose of the options is to provide extra assurance of quality in certain circumstances. It is known that many designers are unaware of the existence of these options, and those that are may not feel qualified to make a judgement on the matter. The majority are of interest primarily to the fabricator and it is important that the choice is left to that party. This applied particularly to chemical control, which can have important implications for avoiding welding problems such as hydrogen cracking, solidification cracking and lamellar tearing. In some instances, where there is general agreement on the advisability of adopting an option, it may be specified in the appropriate execution standard such as EN 1090, which is in the final stages of preparation.
Whilst the specification of an option may improve the assurance of quality of the material and hence the reliability of the structure's service performance, there are two potential penalties. The first is extra costs and the second is an increased risk of delayed delivery due to non-availability, particularly from stockists. This latter point may be more important than the cost of the option itself as one of the benefits of structural steelwork is the speed of project execution from design brief to commissioning of the structure.
From the designer's point of view one of the dilemmas which can confront him/her is whether or not to specify through thickness (TT) testing. This matter is discussed in detail in Section 4.
It is not proposed to discuss the application of the other options, as most are applicable only in special circumstances or where special emphasis on assurance of structural integrity is required in more unusual structures where safety is paramount. In this latter case it is assumed that the designers will be sufficiently knowledgeable to make the necessary choices.
Table 4 Typical cost increases for various quality grades and options
Property type | Property value | Extra cost (1) | Main factor affecting cost range |
Plates | Sections |
Strength grade |
S235/275 |
Basis |
Basis |
|
S355 |
3-7% |
4-13% |
Impact quality |
S420 |
12-17% |
- |
Impact quality |
S460 |
21-34% |
- |
Impact quality |
S550 |
27-43% |
- |
Thickness |
S690 |
37-67% |
- |
Thickness |
S890 |
58-87% |
- |
Thickness |
Impact grade: Effective Charpy test temperature for 27J |
JR +20°C |
Basis |
Basis |
|
J0 0°C |
2-3% |
5-6% |
Strength grade |
J2 -20°C |
3-6% |
7-15% |
Strength grade |
K2,M,N -30°C |
4-8% |
20% |
Strength grade |
M1,NL -50°C |
13-15% |
- |
Strength grade |
Carbon equivalent value (maximum) |
Specified option |
1% |
- |
|
0.02% less than Specified option |
2% |
- |
|
Sulphur (maximum) |
≤0.015% |
1% |
- |
|
≤0.010% |
3% |
- |
|
≤0.005% |
7% |
- |
|
Through thickness tested +UT (S 1 /E 1 ) (2) |
Z15 |
8-15% |
- |
(One plate per cast only) + thickness |
Z25 |
13-20% |
- |
Per cast, batch or plate + thickness |
Z35 |
13-20% |
- |
Per cast, batch or plate + thickness |
Ultrasonic testing (S 1 /E 2 and below) |
t<40mm |
2-4% |
- |
UT quality grade |
4<t<80 |
3-8% |
- |
UT quality grade |
t>80 |
5-12% |
- |
UT quality grade |
(1) Maximum based on current UK market.
- indicates information not applicable or not available.
(2) Ultrasonic testing mandatory prior to TT testing.
1.4 Typical cost data
In order for the designer or fabricator to make a decision on choice of grade or quality option it is useful to know the likely cost implications. Table 4 gives some ideal of the likely additional costs. These are based on current prices available in the UK ex-mill and are expressed in percentage terms. The percentage increases are relative to a lower limit basic cost per tonne averaged across the range of products. In practice there will usually be additional costs depending on order tonnage, sizes (weight, length, etc), transport and other factors. No allowance has been made for these in the percentage increases quoted, so that the percentage values should be considered as upper bounds for material sources from UK mills. Data has not been obtained from stockists, nor from other sources in Europe. Table 4 should be used as an indicative guide to the likely mark up in cost for general design decision making. Where price information is needed for estimating purposes this should always be obtained from appropriate mills or stockists, as pricing policy is likely to be set according to the supplier's individual situation and customers.
The first observation is the low mark up between the 235/275 and 355 strength grades. The values quoted have been based on the same impact grade in each case. The mark up for sections is more than for plate. The extra cost of the higher strengths becomes more significant as the strength exceeds Grade 355. The significance of this data is discussed in Section 2 below.
A similar pattern can be seen for the mark up on impact grade between JR and J2. Again, the mark up for sections is more than for plates. The implications of this are discussed in Section 3 below.
The cost penalties for control of carbon equivalent are insignificant and it is of interest that the optional limits in the current product standards will become mandatory in the forthcoming edition of EN 10025 which will incorporate EN 10113, 10155 and 10137 and contain six Parts.
Apart from the cost extras of the high strength grades, the next most onerous extra occurs if through thickness testing is called up. The significance of this is discussed in Section 4 below.
When considering these cost penalties it needs to be borne in mind that the total cost of the fabricated and erected steelwork will typically be two to three times the material cost.
2. Selection of strength grade
A nominal measure of the relative economy of the different strength grades for design of statically loaded structures can be obtained by reference to the data in Table 4. If the values for plates are used then the strength per unit material cost can be compared to, say, Grade 275 using the following formula:
Table 5 Nominal relative economy of grades based on material cost of plates (based on Table 4)
Strength grade | Relative economy |
Min | Max |
275 |
1 |
1 |
355 |
1.21 |
1.25 |
420 |
1.31 |
1.36 |
460 |
1.17 |
1.38 |
550 |
1.40 |
1.57 |
690 |
1.50 |
1.83 |
890 |
1.73 |
2.04 |
The results show a nominal economic improvement of up to 100% as the strength increases.
For sections the main choice is between Grades 275 and 355 due to the lack of availability of the higher grades. In this case the relative economy of 355 compared to 275 ranges between 1.14 and 1.24.
In terms of the overall economy of the structure these results can be very misleading and it is not uncommon for designers to regret having opted for too high a material grade in some applications. Some of the implications of choosing higher material grades may become apparent as the rules in the design codes become applied. However, there may be disadvantages to the fabricator which can lead to significant increases in fabrication cost, which the designer may not be aware of and which may result in an overall loss of economy or delay to the programme. It is important therefore that all the relevant factors are taken into account at the conceptual stage of the design. Whilst there are some structural applications where the high strength steels are, or could be, used with overall advantage, current practice is to use 355 and/or 275 material for the majority of structural steel applications.
Table 6 lists some of the more important advantages and disadvantages of using higher grade material. The predominance of perceived disadvantages over advantages should not be taken as a message to discount the high strength steels, as there are many applications where the advantages outweighed the disadvantages.
Table 6 Potential advantages and disadvantages of using higher strength grade material
Potential advantages | Potential disadvantages |
A.1 Greater material economy for torsion members and stocky compression members and beams. |
D.1 Loss of overall and local buckling efficiency. |
A.2 Reduced self weight. |
D.2 More stiffening and bracing. |
A.3 Easier erection. |
D.3 More onerous impact requirements (see Section 3 below). |
A.4 Reduced transport costs. |
D.4 Increased deflections. |
A.5 Reduced space loss. |
D.5 Reduced deformability/energy absorption. |
A.6 Reduction in butt welds due to smaller component weights. |
D.6 Increased vibration risk. |
|
D.7 Reduced fire resistance. |
|
D.8 Design more likely to be governed by fatigue (which is independent of grade). |
|
D.9 Less margin for corrosion. |
|
D.10 Less readily available material. |
|
D.11 Increased costs of preparation (cutting, milling, machining, forming). |
|
D.12 Greater time and cost for weld procedure approval. |
|
D.13 Greater difficulty in achieving mechanical property requirements in welded joints. |
|
D.14 High welding residual stress and distortion. |
|
D.15 Higher risk of weld flaws due to chemistry and higher restraint forces. |
|
D.16 Higher inspection scope required. |
|
D.17 Increased fabrication costs and time due to D.10 to D.16 above. |
Unfortunately it is not possible to provide quantified cost or time data for Table 6 as the numbers would vary considerably from one design situation to another. However, it does serve as a useful check list at the conceptual stage, so that the pros and cons can be evaluated and advice sought from suppliers and fabricators before a decision is made.
Examples of structural applications where high tensile steel can be used to advantage are larger span fabricated roof and bridge trusses, where dead weight predominates, fabricated, stocky columns in high rise buildings, medium span replacement bridge beams requiring lifting during possession periods. In such applications the advantages A1, A2 and A3 in particular can often outweigh the disadvantages.
On the other hand use in highway bridge decks and small span railway bridges may be discounted due to fatigue (D.8) where the use of higher stresses will reduce the fatigue life. In some designs there is no real advantage in using a higher grade than 275. In buildings, controls on deflection and vibration of beams may prevent higher stresses being used (D.4 and D.6). In seismic zones, reduced deformability and inadequate fracture toughness may prevent its use (D.5 and D.3). In some masts and towers the increased risk of fatigue and aerodynamic induced vibration may limit the grade used. In some structures such as plate or box girders, where self weight is not an issue, it may be more economical to use a lower grade (say 275) with thicker plates and avoid stiffeners, rather than thinner 355 with stiffeners, so that the saving in fabrication cost can outweigh the small additional material cost.
3. Charpy impact requirements
3.1 Control of brittle fracture - historical development
Brittle fracture is the phenomenon which results in tensile failure of a steel member at stresses below gross yield.
Protection against brittle fracture has been provided by using materials with a minimum level of Charpy impact energy. Historically other specification tests have sometimes been used e.g. Izod, DVM, Schnadt in addition to the Charpy test. However for at least half a century the Charpy V notch test has been used in almost all structural steel product standards for providing a minimum level of fracture resistance. Many of the earlier basic structural steel specifications did not require the Charpy value to be measured, so that reliance had to be placed on an assumed low level of protection for less critical applications. Today all the new European structural grades are supplied to a minimum Charpy impact level.
In the early days of welding brittle fracture became the subject of extensive research particularly in the USA and UK, following notable failures in ships, bridges and pressure vessels. From an assessment of the materials involved in the failures and subsequent testing programmes, very empirical design rules were derived for the minimum Charpy energy required at the minimum expected service temperature. The values were selected on the basis that the material would always be operating with an energy level at the upturn of the transition curve sufficiently above the lower shelf for initiation to be prevented at maximum working stresses. The early recommendations typically varied from 15ft-lbs (20 Joules) to 30ft-lbs (41 Joules) for higher strength materials. For general structural steel product standards containing 'notch ductile' qualities, a value of 20ft-lbs became widely used (27 Joules). Whilst most of the European grades are standardised at 27 Joules, 41 Joules is also used in some cases.
As further understanding of the factors affecting brittle fracture evolved it was realised that specifying 27 (or 41) Joules at the minimum service temperature would not give adequate protection in many circumstances. For this reason the range of notch ductile steels was extended to provide a minimum 27 Joule energy at lower test temperatures, thus effectively providing a higher energy at the minimum design temperature. A typical transition curve for structural steel is shown in Fig.1 showing the likely increase or decrease of Charpy energy with temperature. The particular transition curve in Fig.1 illustrates the typical position of the minimum specification requirement on the transition curve, which is significantly above the lower shelf region.
Fig.1. Form of Charpy transition curve assumed by BS 5400:1982
As the understanding of the theory of fracture improved through the science of fracture mechanics it became clear that quantification of the likelihood of failure risk was more accurately obtained by means of more realistic tests, involving sharper cracks, welded joints and slower strain rates. For very critical applications such as large offshore structure it became customary for specifiers to call for minimum fracture toughness to be confirmed by means of fracture tests such as the crack tip opening displacement test, CTOD, particularly for approval of weld properties. However the Charpy test has remained the criterion in structural steel product standards.
In the UK the much publicised fracture of the Kings Bridge girders in Melbourne, Australia in 1962 [25] , highlighted the need for better protection against this mode of failure in the British Standard Steel Bridge and Building codes. The first 'notch ductile' structural steels with Charpy requirements had already been available from the mid 1950's, but their use was very limited and the design codes which existed did not advise when they should be used. The result was that the majority of structural steel used was not Charpy tested. The other development was the availability of thicker plates and heavier sections. The design rules essentially put maximum limits on the thickness that could be used for the various materials in different circumstances. This was based on the results of fracture test data, in particular full scale wide plate tests. Increasing thickness was known to have a detrimental effect for the following reasons in particular:
- The higher constraint against yielding at the crack tip.
- The ability to develop higher and longer ranging tensile residual stresses.
- The greater risk of large undetected welding flaws being present.
- The greater difficulty in producing fracture resistant steel.
Table 7 Main developments in UK brittle fracture structural design rules (see Section 3.2.5 for explanation of terms)
Date | Code | Application | Main feature | Typical thickness limits for welded Grade 350 steel (mm) (2) at minimum design temperature of -15°C |
No Charpy test | ~J0 equivalent |
1966 |
BS 153 |
Plate girder bridges (UK temperature only) |
Maximum thickness limits introduced |
19 (S) 32 (P)(N) |
50 |
1966 |
BS 499 |
Buildings (Temp ≥ -7°C simple details, no shock loading, no progressive collapse) |
Maximum thickness limits introduced |
40 (S)(P)(N) |
50 |
1982 |
BS 5400-3 |
Bridges |
Design temperature depends on situation. K = 2 for welds and punches holes. K = 4 elsewhere. SCF's reduce thickness limit. Increased thickness where factored stress < 100N/mm 2 |
Not permitted |
55 (P) 27 (S) |
1986 |
BS 5950-1 |
Buildings |
As per 5400-3 (1982) except no SCF reduction |
10 |
55 (P) 27 (S) |
1993 |
Highways Agency rules BD 56/93 |
Highway bridges only |
As per 5400-3, 1982 except: Minimum 18J at T min 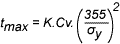 |
Not permitted |
Not permitted (only J2 and above) |
2000 |
BS 5400-3 |
Plate, box and trussed girder bridges |
5 levels of K for different details. 4 levels of stress SCFs clarified. Test temperature not more than 20°C above design temperature. Reduction for high strain rate |
Not permitted |
38 (simple welded) |
BS 5950-1 |
Buildings |
Same basis as BS 5400-3 2000 with some simplification. 4 levels of K. Test temperature not more than 30°C above design temperature. |
Not permitted |
38 (simple welded) |
(1) * limit of specification for Charpy requirement (P) = plates; (S) = sections; (N) = normalised.
The main developments in the UK brittle fracture rules in the last 40 years are shown in Table 7 [7,8,17,18] . Following the Kings Bridge failure, limits were put on thicknesses of the various structural steel specifications currently used at the time, many of which were not designed to be weldable (although a new comprehensive replacement standard BS 4360 for weldable steels was shortly to replace the old standards). Limiting thicknesses were placed on non-impact tested based on typical expected properties. The main differentiation was between as-rolled products (thin plates, flats and sections) and the normalised thicker plates. Most of the impact grades had guaranteed Charpy properties only up to 50mm thickness (unlike today where, for example, the non-alloy steels in EN 10025 are guaranteed for 250mm for plates and 100mm for sections - the latter covering most the current range anyhow - see Table 1).
In 1982 the rules for bridges were amended in the new limit state bridge code BS 5400, Part 3. [22] A simple consistent relationship was provided covering all the grades and Charpy qualities. The allowable thickness in millimetres was equal to twice the Charpy energy in Joules at the minimum design temperature for Grade 355 steel for welded construction and punched holes; otherwise the factor was four times. The transition curves shown in Fig.1 were used to derive the thickness limits for different design temperatures. The thickness requirement was also inversely proportional to the yield strength (see formula given in Table 7). For the first time non-impact tested qualities were prohibited for bridges.
Other new features were the increase in permitted thickness for regions of lower tensile stress and a reduction in thickness where potential fracture initiation sites were in regions of high geometric stress concentration. The importance of the situation was also reflected in the rules for pressure vessels and offshore structures developed in the 1980s, where the presence of geometrical stress raisers such as nozzles and unstiffened tubular nodes were known to increase the likelihood of fracture. This is reflected in the case history of brittle fractures in service where the majority either originate from gross geometrical discontinuities and/or from serious welding flaws. For simple welded construction the thickness limits were not very different from those derived in the 1960s. The same rules were applied to the new limit state building code BS 5950-1 in 1986. The only significant difference was that, due to the widespread use of small to medium rolled sections which were from non-impact tested material it was decided to allow their continued use, but in smaller thicknesses than previously.
In the early 1990s concern was expressed about the potential use of steel with energies close to the lower shelf. The Highways Agency produced amendments to some of the criteria used in the bridge code. A minimum nominal energy limit of 18 Joules was imposed for highway bridges, which at minimum design temperatures of -15 to -20°C meant that the JR and J0 qualities could be used. This was not seen to be a serious economic penalty (see Table 4).
The other concern was whether the energy requirements for the strength grades above 355 were adequate, although their use was very low. It was therefore decided to use an inverse square relationship to replace the linear one used in BS 5400. The square relationship appeared to be more consistent with theoretical principles perceived at the time and it was considered that its use would also remove some unnecessary conservatism from the rules for the 235 and 275 grades.
In 1995 the Highways Agency decided that the whole basis of the fracture rules should be reviewed. A programme of work was carried out which resulted in a comprehensive revision in both the bridge and building rules in 2000. The background to these rules is discussed below.
3.2 Background to new UK brittle fracture design rules
3.2.1. Main objectives
A number of developments in the science of fracture behaviour had taken place since the 1980s rules were published. The prime objectives of redrafting were:
- To take account of the developments in fracture mechanics method which had taken place in the revisions of PD 6493:1980 (recently reissued as BS 7910).
- To take account of recent developments by K Wallin and the resulting 'master curve' for correlating Charpy test data with fracture toughness. [23]
- To review the assumptions regarding worst flaw dimensions, and to include consideration of fatigue crack growth.
- To rationalise the treatment of stress concentrations and to provide more user friendly rules for the designer.
- To provide a more consistent level of probability of failure over the full range of structural details.
3.2.2 Basic principles
The fundamental principle of preventing brittle fracture is to ensure that the following condition holds good at every potential initiation site:
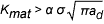
where:
K mat is the fracture toughness of the material at the crack tip.
α is a coefficient depending on the severity of the local stressing conditions at the crack tip.
σ is the maximum tensile stress taking account of both applied and residual stresses (usually taken to be f y ( R eH or R e0.2 )).
a d is the effective height of the crack.
Whilst the expression looks simple in principle, the number of independently varying parameters involved is considerable when it is applied to real structural situations. Many of these are difficult if not impossible to control economically. This results in a very wide range of potential scenarios, which the designer cannot hope to address individually. For this reasons substantial simplifications, guesses and assumptions have to be made in drafting fracture prevention rules. It is not surprising therefore that there tends to be a wider variation of rules in different codes than is the case with more predictable and controllable limit states such as buckling or ductile rupture.
Table 8 illustrates the main factors affecting the probability of fracture, together with the three main parties responsible for determining the quantity or quality of the various parameters. It will be seen that the responsibility for control is shared fairly equally between the designer, steel maker and fabricator/erector.
Whilst the designer is responsible for controlling the applied stresses and determining the dimensional details, the severity of residual stresses will be entirely out of his/her control. In exceptional cases with heavy congested weldments such as cable anchorages in large bridges it may be economical to use stress relief, but this is usually impractical for most heavy structural members.
High levels of residual stresses will be induced in plates and sections at the rolling mill. Cutting and straightening will introduce more, as will jigging and welding. As sub-assemblies are joined to form larger structures on site the potential for introducing longer range as well as further local residual stresses increases, due to fit up errors, temperature differentials and high restraint, etc. This applies whether the site joints are welded or bolted. Control of the final state of residual stress by the fabricator or erector is a practical impossibility in most structures. On the other hand some degree of control of notch like features is practicable and essential to avoid fracture. Sharp planar welding flaws such as cracks and lack of fusion must be controlled by the fabricator through selection of steel chemistry, choice of welding procedure and welder qualification. Cracks or notches arising from poor cutting procedures or worn hole punches must also be controlled by the fabricator. The designer can have some influence by ensuring that partially penetrated joints are not used inadvisedly, good welding access is possible, appropriate weld quality limits are specified and that an adequate scope of inspection is insisted upon.
The other factor which is very much in the control of the designer is the risk of fatigue crack growth, particularly from unspecified notches. The UK fatigue rules and workmanship specification for bridges (BS 5400 Parts 10 and 6 respectively) have recently been amended to reflect the importance of controlling flaws in fatigue prone structures. Some of the higher fatigue design strengths have been reduced to take into account the difficulty of detecting and assessing small flaws by NDT. The quality requirements and extent of inspection are now related specifically to the degree of cyclic stressing at the detail concerned. [24] More lightly cyclically stressed areas have to be identified by the designer. This follows the principles adopted in ISO 10721-2 (fabrication of steel structures). [9]
Table 8 Main factors influencing brittle fracture and parties responsible for control
Factors influencing fracture | Symbol | Main controlling party |
BS | EN | Designer | Steel manufacturer | Fabricator/erector |
Stress intensity K I |
Peak tensile stress |
Applied stress field |
σ |
σ ED |
✔ |
✔ |
✔ |
Residual stresses (long range and local) |
σ R |
σ s |
( ✔) |
( ✔) |
✔ |
Stress raisers geometrical, local |
K,Y,M k |
|
✔ |
|
( ✔) |
Notch severity (Flaws, crack-like features) |
Height |
a |
a |
( ✔) |
|
✔ |
Length |
l |
l |
( ✔) |
|
✔ |
Shape |
|
|
( ✔) |
|
✔ |
Orientation |
|
|
( ✔) |
|
✔ |
Distance from surface |
|
|
( ✔) |
|
✔ |
Sharpness |
|
|
|
|
✔ |
Growth by fatigue |
da/dN |
da/dN |
✔ |
|
|
Initiation fracture toughness, K mat |
Minimum temperature |
|
U, T min |
T ed |
✔ |
|
|
Thickness |
|
t |
t |
✔ |
|
|
Rolling direction |
|
|
|
✔ |
|
( ✔) |
Microstructure (grain size, and orientation, ageing) |
Steel making process |
|
|
|
✔ |
|
Chemical analysis |
|
|
|
✔ |
|
Segregation |
|
|
|
✔ |
✔ |
Welding consumables |
|
|
( ✔) |
|
✔ |
Welding/cutting procedures |
|
|
|
|
✔ |
Thermal history |
|
|
|
✔ |
✔ |
Strain history |
|
|
|
✔ |
✔ |
Strain rate |
|
⋅ |
⋅ |
✔ |
|
|
Key: ✔ = Major role ( ✔) = Minor role. |
On the material side of the equation the fracture toughness will be dependent on the minimum service temperature, the thickness, rolling direction and strain rate, which are essentially determined by the designer. As far as the metallurgy is concerned the parent steel will be primarily controlled by the rolling mill. The material will be impact tested by taking Charpy test pieces from one item per cast or lot. In general the locations will not sample the regions of lowest toughness (near the centre of continuously cast plates and in the region of the web-to-flange junction in sections). At these positions the effects of segregation, thermal and strain history can result in an adverse shift of transition curve of 40° or 50°C in severe cases.
It is normal for specifications to call for weld metal and HAZ Charpy properties to match the minimum requirement for the parent metal. Whilst this is normally provided by procedure qualification testing, normal deviations in consumables and application of the weld procedures can result in shortfalls in weld toughness.
Typical distributions of fracture toughness in parent materials and welds are normally very wide, so that the average fracture toughness is likely to be well above the specification limit. BS 5400 Part 6 requires that a proportion of transverse butt welds in plate girder flanges have coupon run-off plates so that the mechanical properties can be verified in production.
Given the wide range of variables and the limited degree of control over many of them, the drafting of appropriate fracture rules requires a good practical knowledge of all these aspects (including design, materials and workmanship) to be sure of reaching a sound engineering solution, which is practicable to apply and strikes the right balance between economy and undue conservatism. The starting data are as important as the application of the fracture mechanics calculations.
3.2.3 Input data for scenarios assessed
- Structural geometries
Plate with various stress concentrating details e.g. welded cover plate, gussets, transverse stiffeners, width 1000mm, thickness up to 150mm.
- Applied tensile stresses
σ Ed =0.75 σ y and 0.25 σ y membrane
- Residual stresses
As-welded σ s = σ y
- Flaw locations and dimensions
Weld toe: a=0.15 t, a min = 3mm, a max = 12mm, l = 100mm, surface breaking semi elliptical.
Cut edges, holes: lower values of a assumed.
- Yield strengths
As per EN Product Standards with adjustment for thickness.
Yield strength increased for reduced temperature.
Range investigated 235 to 460.
- Fracture toughness
Wallin C v to K mat correlation used with a probability level of 0.4.
Minimum specified Charpy value from EN Product Standard (adjusted to 27J for other energy requirements).
Reduced fracture toughness assumed for butt welded joints between rolled sections.
- Fatigue growth
Where cyclic stresses occur, the initial flaw is assumed to be smaller than in iv) above, so that at the end of the design life there is a 50% probability that the final size is equal to that in iv) above.
- Strain rate
Statically loaded structures ⋅ =10 -4 s -1
Impact loaded structures ⋅ =10 -1 s -1 .
- Minimum service temperatures
From 0°C to -50°C.
3.2.5 Analysis and results
The method used for assessing the test temperature for a 27 Joule Charpy energy for each scenario is described in Appendix A. The analysis was deterministic in that no attempt was made to ascribe probabilities to the key parameters, apart from selecting the K mat /Charpy correlation probability value of 0.4. This latter value is used as a final adjustment to correlate the results with previous service experience. In making this engineering judgement, account was taken of the fact that in practice it was known that there was a high probability that the actual Charpy energy measured on modern steels is generally well above the minimum required by the product standard, with average values typically in the region of 100 Joules.
From these calculations, tables of limit thicknesses were derived for different service temperatures, Charpy 27 Joule test temperatures, applied stress levels, yield strengths, residual stress levels, and details with different stress concentrations. The latter were analysed using elastic and elastic-plastic three dimensional finite element analyses.
It was found that the results could be expressed with an acceptable degree of accuracy by the following simple relationship:
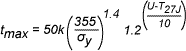
where:
t max is the maximum permissible thickness in millimetres.
σ y is the nominal yield strength of the strength grade considered.
U is the minimum design temperature in °C.
T 27J is the Charpy test temperature for the impact quality considered.
k is the product of four factors as shown below:
k = k d x k g x k σ x k s
where:
k d is a factor between 0.5 and 2 which depends on the construction detail at the site of potential fracture initiation being considered.
k g takes account of any gross geometrical stress concentration in which the detail is sited.
k σ takes account of the maximum applied tensile stress level.
k s takes account of the strain rate.
Table 9 BS 5400 Factor k d
(Note - the full standard should be consulted for complete definitive requirements)
k d values |
Type of construction | Product forms | Detail description (see also Fig.2) | k d * |
Potential fracture initiation site | BS 5400 Pt 10 Detail type |
Non-welded part |
All |
As-rolled surface Ground or machined edge |
1.1, 1.2, 1.3 |
2 |
Flame cut edge Mechanically fastened joints (drilled or reamed holes) |
1.4 1.5 to 1.11 |
1.5 |
Mechanically fastened joints (punched holes) |
Where permitted |
1 |
Welded part |
All |
Longitudinal attachment welds on built up members Transverse weld toes at ends of short welded attachments |
2.1 to 2.5 2.9, 2.10 |
1 |
Transverse weld toes at ends of long welded attachment (1 > 150mm) |
Narrow (w ≤ 50mm) |
2.6 |
0.7 |
Wide (w > 50mm) |
2.7 |
0.5 |
On edge with unradiused ends |
2.11 |
Plates |
Transverse butt welds between single plates |
3.1 to 3.4, 3.5 |
1 |
Transverse butt welds between members built up from plate |
3.6, 3.7, 3.8, 3.9, 3.10 |
0.7 |
Sections |
Transverse butt welds |
3.6, 3.7, 3.8, 3.9, 3.10 |
0.5 |
All |
Fillet welded cruciform and tee joints |
3.11 |
1 |
One of the major objectives was to assist the designer to distinguish between details where the stress concentration was covered by k s and those where a geometrical stress concentration factor applied. This was done by making use of the fatigue classification tables in BS 5400 Part 10, which had already been used by bridge engineers for some twenty years. These illustrate the main types of detail found in bolted and welded steel construction.
A summary of the k d factors is given in Table 9 and the detail types are illustrated in Fig.2. The latter show the lower part of the fatigue classification tables. The k d values are indicated on the figures and this shows how the values tend to follow the same general trend as the fatigue strengths, which is not surprising, considering that both are heavily dependent on local stress concentration effects. Stress concentrations such as those found at bolt holes, ends of cover plates, gussets, etc are taken into account by the k d factor. The end-to-end butt welding of section (type 3.6) does not involve a high local stress concentration due to the joint geometry. However there is a significant risk of non-detected lack of fusion in the junction region (which is why the design fatigue stress is low). This is also an area in sections where low toughness is likely to be found, as discussed earlier. Hence the value of k d is 0.5. The range of values from 0.5 to 2 shows how important is the detail when considering fracture avoidance.
Fig.2. Illustration of k d detail factors and features requiring k g factors
Key:- ( ) Higher classifications which may be used if special high levels of workmanship above those specified in Part 6 of this British Standard are achieved.
- * k d = 0.7 if fabricated from plate
- Δ σ c = fatigue design strength (N/mm2 at 2 x 106 cycles); B, C ... W - BS5400 Pt.10 designations
Where geometrical stress concentrations have to be taken into account as well, these are indicated by a double asterisk in Table 9 and are also noted in the fatigue classification table. The value of k g has to be obtained from:
k g = K -0.5
where K is the ratio of the peak principal stress at the initiation site to the nominal stress σ Ed at the same cross section and is derived from standard solutions or by finite element analysis. This applies to large changes of section, e.g. access holes, sudden changes of width or unstiffened joints between open or hollow sections (see Fig.2 for some typical examples). For example, an initiation site at the highest stress position on a large circular opening, where the SCF was (say) 3, would have a k g = K -0.5 factor of 0.58.
Table 10 BS 5400 Factor k σ
(Note: The full standard should be consulted for complete definitive requirements)
k σ values |
Stress limits | k σ |
k d > 0.7 | k d ≤0.7 |
σ max > 0.5 σ y in tension |
1 |
1 |
0.25 σ y < σ max ≤ 0.5 σ y in tension |
1 |
1.25 |
σ max ≤ 0.25 σ y in tension |
1.5 |
1.5 |
All stresses compressive |
2 |
2 |
* Note - k d values for welded parts may be increased by 50% if all welds have received full stress relief by post weld heat treatment |
The effect of maximum applied stress level on k σ can be seen in Table 10. This shows a benefit of 1.5 on thickness where low tensile stresses apply (for example in columns). Where the calculations show that the in-service stresses are nominally compressive there is still a requirement to provide a level of protection and this has been provided by a k σ factor of 2. The reasons for this are threefold:
- During erection some 'compression' members may be stressed in tension (often highly) and are also at more risk of shock loading.
- Slender struts and compression panels will sustain high out-of-plane bending moments due to significant second order geometry changes. These can easily put the extreme fibres into tension. This does not normally appear in the designer's calculations.
- There is a requirement in UK design codes to provide robustness against progressive collapse from low probability events such as accidental impact, explosions, etc.
The two cases of strain rate considered give a k d of 1 for normal loading as in bridges and buildings and a k d of 0.5 where impact conditions apply, for example the fixings of bridge parapets or columns in multi-storey vehicle parks.
The resulting thickness limits for the most common conditions are shown in Table 11. These are for k=1; for example, high tensile stresses, simple welded detail, no geometrical stress concentration and no impact. The results show that there is a relatively modest change in thickness requirement with temperature. For example, the allowable thickness reduces by about 30% as the minimum design temperature drops by 20°C, (or the test temperature rises by 20°C). This is a direct influence of the Wallin K mat /Charpy relationship.
Table 11 BS 5400 (Bridges) and BS 5950 (Buildings) thickness limits for details where k = 1
Strength grade | Impact quality | Effective Charpy test temperature, T 27J | Maximum permitted thickness (mm) according to design minimum temperature U in °C (1), (2) |
U = 0 | -5 | -10 | -15 | -20 | -25 | -30 | -40 | -50 |
S235 |
JR |
+20 |
0 (62) |
0 (38) |
0 (17) |
0 |
0 |
0 |
0 |
0 |
0 |
J0 |
0 |
89 |
81 |
74 |
68 |
62 |
0 (38) |
0 (17) |
0 |
0 |
J2 |
-20 |
128 |
117 |
107 |
98 |
89 |
81 |
74 |
62 |
0 |
S275 |
JR |
+20 |
0 (50) |
0 (30) |
0 (14) |
0 |
0 |
0 |
0 |
0 |
0 |
J0 |
0 |
71 |
65 |
60 |
54 |
50 |
0 (30) |
0 (14) |
0 |
0 |
J2 |
-20 |
103 |
94 |
86 |
78 |
71 |
65 |
60 |
50 |
0 |
N.M |
-30 |
124 |
113 |
103 |
94 |
86 |
78 |
71 |
60 |
50 |
NL, ML |
-50 |
178 |
162 |
148 |
135 |
124 |
113 |
103 |
86 |
71 |
S355 |
JR |
+20 |
0 (35) |
0 (21) |
0 (10) |
0 |
0 |
0 |
0 |
0 |
0 |
J0 |
0 |
50 |
46 |
42 |
38 |
35 |
0 (21) |
0 (10) |
0 |
0 |
J2 |
-20 |
72 |
66 |
60 |
55 |
50 |
46 |
42 |
35 |
0 |
K2, N, M |
-30 |
86 |
79 |
72 |
66 |
60 |
55 |
50 |
42 |
35 |
NL, ML |
-50 |
124 |
114 |
104 |
95 |
86 |
79 |
72 |
60 |
50 |
S420 |
N, M |
-30 |
68 |
62 |
57 |
52 |
47 |
43 |
40 |
33 |
27 |
NL, ML |
-50 |
98 |
90 |
82 |
75 |
68 |
62 |
57 |
47 |
40 |
S460 |
Q |
-20 |
50 |
46 |
42 |
38 |
35 |
32 |
29 |
24 |
0 |
N, M |
-30 |
60 |
55 |
50 |
46 |
42 |
38 |
35 |
29 |
24 |
QL |
-40 |
72 |
66 |
60 |
55 |
50 |
46 |
42 |
35 |
29 |
|
NL, ML |
-50 |
87 |
79 |
72 |
66 |
60 |
55 |
46 |
42 |
35 |
|
QL1 |
-60 |
104 |
95 |
87 |
79 |
72 |
66 |
60 |
50 |
42 |
Notes: (1) Bracketed values apply to buildings only (2) Some thickness values may be above limits of EN steel product specifications. |
The more important influence is the effect of yield strength. For example, the allowable thickness drops by 60% if the grade is raised from S235 to S460 for the same Charpy test temperature (e.g. J2 and Q respectively, which are both tested at -20°C). This is a direct influence of the 1.4 exponent in the basic thickness relationship above.
The other important issue is to what extent material should be used at temperatures well below the Charpy test temperature. Table 11 shows that for bridges a cut off has been applied to all minimum design temperatures 20°C lower than the test temperature. This is less severe than the 1993 rule for highway bridges (see Table 7), but it still means that for practical purposes JR is not useable for bridges. for buildings a linear 'ramp down' has been applied from the full thickness at 20°C below the test temperature to zero at -35°C. This enables the large volume of light to medium sections in buildings to be used either in the JR or J0 qualities (see Table 1). The choice of limit cut off (or ramp down) on temperature differential between test and service is essential to guard against material with steep transition curves being used under low shelf conditions.
From the designer's point of view, the use of a factor on thickness has always been a convenient way of determining the required impact quality. An alternative way is to make any adjustments on the basis of test temperature. For example, a downwards shift of service temperature of approximately 40°C (or an upwards shift of test temperature of 40°C) results in a having of the allowable thickness. This system has not been adopted for two reasons. Firstly, the temperature shift still has to be converted to an allowable thickness. Secondly, there is a greater risk of serious errors occurring due to the use of the wrong sign being inadvertently used.
From the table it can be seen that for steel enclosed in buildings, when the minimum design temperature is taken as -5°C in the UK, the main bulk of the sections can be used for welded construction, as the thickness limit is 30mm for S275 and 21mm for S355 (see Table 1). Even at -15°C, which is the normal exposed minimum design temperature in the UK, some of the thicker sections will need to be J0 unless the tensile stresses are low or the high tensile stress positions are unwelded or bolted. As can be seen from Table 4 the cost penalty is only a few percent on material only. The most important issue is availability. As steel making practice continues to progress, it would improve the availability issue considerably if all structural steels could be produced to J0, or better still J2 as a minimum. It is almost certain that the small penalty of extra production cost would be compensated by reducing the substantial range of product variations.
The other effect of the new rules is to make designers more aware of the effect of using details containing high stress concentrations. The new UK rules represent a significant improvement in consistency of probability of failure across the full range of design situations, without making the process of selection of impact quality any more difficult to carry out in the design office.
It should be noticed that the UK rules are for the design of new buildings and bridges of normal construction in materials up to grade S460. They are not intended to cover the assessment of existing structures, where fitness-for-purpose principles should normally be adopted.
3.3 European brittle fracture rules
3.3.1 Historical development
Considerable effort has been made over the last 15 years to develop common brittle fracture design rules for all steel structures. The main developments of the rules are listed in Table 12.
Table 12 Development of Eurocode 3 brittle fracture rules
Date | Document | Scope | Main features | Typical thickness limits for Grade S355 in tension at minimum design temperature of -15ӼC (mm) |
Application | Steel grades | Detail types | Stress differentiation | Other | JR | J0 |
1992 |
ENV 1993-1-1 |
General steelwork |
235 to 355 |
3 (non-welded complex stress concentration regions) |
2 (tension, compression) |
2 loading rates (static, impact) 2 consequences of failure |
25 (non-welded) 8 (welded) |
62 (non-welded) 18 (welded) |
1994 |
ENV 1993-1-1-A1 |
As per ENV 1993-1-1 |
420 and 460 only |
3 (non-welded complex stress concentration regions) |
2 (tension, compression) |
2 loading rates (static, impact) 2 consequences of failure |
Not applicable |
Not applicable |
1997 |
ENV 1993-2 |
Bridges only |
235 to 460 |
1 (no differentiation |
1 |
Adjustment for loading rate. Fatigue allowed for by in-service inspections |
Not included |
60 (all details) |
2003 |
EN 1993-1-10 Final Draft |
General steelwork |
235 to 690 |
1 (no differentiation) |
3 tensile levels |
Adjustment for loading rate. Adjustment for cold forming. Adjustment for 'safety' (not quantified). Adjustments use temperature shift. Fatigue allowed for by in-service inspections |
22 (all details) |
37 (all details) |
The first draft rules (1992), covering all structural steelwork, had various differentiations including three types of detail, but only up to Grade 355. [13] The limiting thicknesses for J0 quality were below those the limits allowed in the current UK rules for welded steelwork (see Table 27 1982 and 1986). In 1997 the draft Part 2 for Bridges was produced. [14] This eliminated the differentiation on the basis of detail type and stress level, but added a formula for calculating the effect of loading rate. Another addition was the recommendation that if fatigue was likely to occur then in-service inspection would be necessary. In other words, it would not be possible to rely on safe life design, as had been the principle adopted in the UK. The method of calculation of the thicknesses was revised, leading to an increase of above three times over the rules (ENV 1993-1-1).
Following the publication of the draft codes (ENVs) Sub-Committee 3 (steel) of CEN Technical Committee 250 (Eurocodes) decided to change the document system. As a result brittle fracture became a separate Part as an EN, the number being 1993-1-10 (-1.9 covering fatigue). These documents cover all structural steel applications. [15,16]
Part 1.10 has now been approved by CEN/TC250/SC3, though not without some difficulty as the next section will explain.
3.3.2 Comparison of the current EN and BS rules
The common features between the two rules are:
- The general trend of the thickness versus temperature relationships are similar particularly for Grade S355 (see Table 13). This is no doubt due to the fact that the same Wallin criteria have been used for the K mat /Charpy value correlation. Some differences are due to the rounding of the EN values to the nearest 5mm.
- The same criteria have been used for estimating the effect of loading rate.
- The thickness limits for the different grades and impact qualities are provided in a convenient table against minimum design temperature as in Table 11 above.
Table 13 Comparison of EN and BS Codes - variation of thickness with temperature
Code | Maximum thickness (mm) according to minimum design temperature, °C |
10° | 0° | -10° | -20° | -30° | -40° | -50° |
EN 1993-1.10 |
90 |
75 |
60 |
50 |
40 |
35 |
25 |
BS 5400-3 for k=1 only |
86 |
72 |
60 |
50 |
42 |
35 |
0 (21) |
The most significant differences are as follows:
In the EN document, there is no differentiation between detail types or rules for dealing with gross stress concentration effects in the EN code.
Table 14 shows the differences that would exist for, say, Grade 355 at a
T min of -20°C in full tension. This is a very substantial difference and EN 1993 Part 1.10 was only able to achieve a fully positive vote on condition that National Bodies could introduce an adjustment through the provisions of a National Annex.
Table 14 Comparison of thickness limits (mm) for differences in detail types (Grade S355 J2)
Detail type | BS 5400 Part 3 | EN 1993-1.10 |
As rolled or machined edge |
100 |
50 |
Flame cut edge or drilled holes |
75 |
50 |
Simple welds, punched holes |
50 |
50 |
Ends of longitudinal stiffeners |
35 |
50 |
Ends of cover plates, welds between sections |
25 |
50 |
Ends of cover plates at geometric SCF of 3 |
14 |
50 |
The effect of yield stress is shown in Table 15 for a T 27J of -20°C (J2), a T min of 20°C and k=1 and full tension. This results in an inverse linear relationship for the EN as opposed to the 1.4 power for the BS. This difference is thought to be mainly due to the reputed assumption of residual stresses equal to 100N/mm 2 having been used for the derivation of the EN values. This compares with yield stress in the BS method.
Table 15 Comparison of thickness limits (mm) for differences in yield strength
Strength Grade S | S235 | S275 | S355 | S460 | S690 |
EN 1993-1-10 |
75 |
65 |
50 |
40 |
25 |
BS 5400-3 |
89 |
71 |
50 |
35 |
(20) |
( ) extrapolated using the BS 5400 formula |
The effect of the tensile stress level is shown in Table 16 for three different grades for a T 27J and T min value of -20°C (J2). It can be seen from these results that there is a very substantial difference at the high strength end of the table. The fact that the EN allows nearly three times the thickness is of some concern. It is likely that the assumption of residual stresses equal to 14% of yield, as opposed to 100% of yield in the BA case could have been the main cause of this difference.
Table 16 Comparison of thickness limits (mm) according to stress level for J0 and T min = -20°C
Grade | Full tension | 0.25 yield | Ratio  |
235 |
EN |
75 |
155 |
2.1 |
BS |
89 |
134 |
1.5 |
355 |
EN |
50 |
130 |
2.6 |
BS |
50 |
75 |
1.5 |
690 |
EN |
25 |
85 |
3.4 |
BS |
(20) |
(30) |
(1.5) |
( ) extrapolated using the BS 5400 formula |
The effect of cut off assumptions can be seen in Table 17 which compares the thickness limits for S235JR which has a T 27J value of +20°C for full tension and k=1. The EN allows mild steel to be used at 70°C below the T 27J test temperature in a thickness of 20mm without any restriction on stress raising conditions.
Table 17 Comparison of thickness limits (mm) for JR quality mild steel for k=1
T min | 0° | -10° | -20° | -30° | -40° | -50° |
EN 1993-1.10 |
50 |
40 |
35 |
30 |
25 |
20 |
BS 5400-3, (BS 5950-1) |
0 (62) |
0 (17) |
0 |
0 |
0 |
0 |
( ) Buildings onl |
Different assumptions have been used for the assumed flaw size in relation to thickness size used for the two codes shown in Table 18. In many of the drafts the values for the EN were based on the initial height 'a' being equal to the natural logarithm of the thickness in millimetres (see Table 18). However, recent drafts have suggested that 50% of this value was also used. This illustrates a major difference in principle adopted for the two codes. In the EN the flaw is assumed to grow under fatigue. This can lead to problems of excessive crack size before the end of life, hence the need to require in-service inspection. The UK rules are based on limiting fatigue classifications so that much smaller flaws are eliminated at the fabrication stage. The cumulative costs of repeated in-service inspection (including access and paint removal) can be two orders of magnitude more expensive than one final inspection after fabrication. It should further be pointed out that however tough the steel is, if extensive fatigue cracks are allowed to grow in-service the member will fail by plastic collapse after a short time anyhow.
Table 18 Assumed reference crack height and length for brittle fracture rule derivation
Plate thickness t (mm) | 12 | 25 | 50 | 100 |
Static structures |
EN 1993-1.10 |
a x l |
2.3 x 12 |
3.2 x 16 |
3.9 x 20 |
4.6 x 23 |
BS 5400-3 |
a x l |
3 x 100 |
3.8 x 100 |
7.5 x 100 |
12 x 100 |
Fatigue loaded structures |
EN 1993-1.10 |
a x l |
Greater than static (values not known) |
BS 5400-3 |
a x l |
As for static |
The above comparisons illustrate that differences of approach in scenario selection and assessment method can make significant differences to the Charpy selection rules.
4. Through thickness testing
4.1 The need for testing
Historically, there have been two reasons for through-thickness testing. The first has been to ensure that gross laminations are not present in the material, particularly in plate as these can be extensive enough to result in a reduction of buckling strength. In regions of high shear or though-thickness tension such as in some tee and cruciform joints, laminations can result in structural weakness. Fortunately, steel making practice has improved considerably and this risk is less today that it was half a century ago. In the days of riveted joints the clamping action of the rivets tended to reduce or eliminate the structural weakness.
When welding of such joints became the norm, another risk emerged - that of lamellar tearing. This phenomenon occurred in the same joint types particularly when welded under conditions of high restraint. It resulted in step-like cracking along the rolling plate of the material across which the thermal weld shrinkage stresses were taking place. It could easily occur in material which appeared to be relatively clear of planar rolling discontinuities.
4.2 Method of control
In the UK, the methods used to protect against these risks tended to be left mainly to the fabricator. However, in BS 5400 Part 6, the UK specification for materials and workmanship in bridges, it is a requirement to check critical regions of plates, such as web to flange junctions, bearing stiffeners, cruciform joints, etc, using ultrasonic compression probe testing to specified quality. The decision as to whether to pay extra for the entire plate to be tested at the mill, or whether to test the plates locally at the critical regions at the fabrication shop and cut the plates accordingly to keep poor quality areas away from critical weld locations, was left to the fabricator.
The same principle applied to lamellar tearing, which is essentially a welding problem. The fabricator has been made aware of the risks in the UK welding code BS 5135 for many years and guidance on avoidance is provided. [10] Similar advice has been incorporated into EN 1011-2, the European successor to BS 5135, (see Annex F of EN 1011-2) which contains six pages of guidance. [11]
The three main methods of control of lamellar tearing are:
- To use clean steel, particularly with respect to sulphur.
- To specify through-thickness testing.
- To select welding procedures to reduce constraint during welding and to use low yield strength consumables to 'butter' the surface of the susceptible material.
In the structural industry, it has been the practice to leave the decision to the fabricator as to which of these options to adopt. The only shortcoming of this approach is that a decision needs to be made prior to the tender stage so that the extra costs can be allowed for. These are not inconsiderable (see Table 4).
In the offshore industry, where heavy tubular nodal construction commonly involves tee (and sometimes cruciform) joints with full penetration butt welds 50mm thick or more, it has been common practice to specify through-thickness tested material as standard. Current rolling practice has benefited from this market as a result, with most European mills providing clean steels with sulphur limits generally lower than 0.01% and often less than 0.005%. The guidance in EN 1011-2 Annex F shows that with these levels of cleanliness there is a high probability that the material will have good through-thickness properties, and will probably meet the Z25 or Z35 short transverse reduction of area requirements (STRA) as a matter of course. [21] As a result, structural fabricators purchasing their materials from reliable sources do not often need to pay extra for confirmatory testing to be carried out. This practice has resulted in very few problems of lamellar tearing in the structural industry in recent years.
This does not mean to conclude that there may not be a place for ordering steel to be tested for a specific Z quality in some circumstances, as in the offshore practice.
4.3 The role of the designer
There has been discussion for some time in the UK as to whether designers should also be guided towards specifying 'Z quality' (though-thickness) testing in certain circumstances. So far, this has never been adopted in the UK steel building or bridge code. The main argument has been that, like control of hydrogen cracking and the specifying of minimum carbon equivalent value, it is a welding quality problem and therefore a matter for the fabricator to decide in the light of the particular material sources, welding process and procedures he uses.
The prime assurance that the designer has that the structural integrity will not be compromised by lamellar tears is to specify that an adequate NDT regime is implemented to ensure that such flaws are not left in the final structure. It is of concern that the draft European specification for the execution of steel structures ENV 1090-1 does not contain any minimum requirement for final NDT of structural welding. Reliance on weld quality in the end product cannot be left entirely to the qualification of consumables, procedures and welders. However, good the quality assurance system is, there is always a risk of adverse deviations in the many factors which influence weld quality, causing flaws to be left in the work. NDT is the last safety net.
ISO 10271, which has already been referred to, has recommendations for minimum levels of NDT depending on the joint configuration and thickness. One of the factors in determining these recommendations was the risk of lamellar tearing. Both the UK steel building code and the bridge code (BS 5950 Part 2 and BS 5400 Part 10), have minimum mandatory NDT specifications for production. These are essential to specify in codes, as many designers do not have the knowledge or experience to judge the suitable extend of NDT, with the result that over- or under-specifying can easily occur.
On the Eurocode scene, the final draft of Eurocode EN 1993-1-10, which contains the brittle fracture rules, also contains detailed advice on selection of Z quality levels. A detailed formulation is given which quantifies aspects such as joint type, weld size, material thickness and degree of restraint and converts these into a number which is a measure of the Z quality required to prevent lamellar tearing. Whilst the general trends of the formulation appear to be in the right direction, there are some parameters which are debatable. For example, there is emphasis on the cross plate thickness in tee and cruciform joints, but not on the incoming plate thickness or size of the butt weld throat. It is also suggested that preheating is beneficial, which in restrained conditions may not be the case.
The worrying aspect of the guidance in EN 1993-1-10 is that a large proportion of structural steelwork could be judged to require at least Z15 quality. For example, a plate girder with full or partial penetration web to flange welds with flanges say 30mm thick and webs 12mm thick would require Z15 tested material, according to the formulation. With normal modern sulphur-controlled steels this would be an unjustifiable waste of money. More importantly if the same joint was to be used between a beam and column in buildings, the availability of the column material with such a specification could pose a serious problem.
It has been proposed that this guidance material is transferred to EN 1011-2 Annex F and that EN 1993-1-10 limits itself to defining only joint conditions where Z35 and above would be required. For example, tee joints with butt weld throats in excess of (say) 35mm or cruciform joints with butt weld throats in excess of (say) 25mm. As the final draft still contains this formulation, if designers apply the test requirements accordingly, there could be a severe penalty to the economy of steel construction.
5. Conclusions
The paper has reviewed the wide range of structural steel products and qualities available today for civil construction. Current UK and European product standards and design code developments regarding the selection of materials have been discussed.
The main conclusions are:
- The choice of products and material qualities available for steel construction is extensive.
- It is important that designers consult fabricators and steel suppliers before deciding on some of the ordering options available.
- Availability may be as important a factor as cost.
- Caution needs to be exercised when exploring the benefits of moving to high strength steels for general structural use.
- There may be an economic case for moving from JR to J0 or J2 impact quality for the base level of general structural steel.
- Development of Charpy selection rules is a complex issue and there are still some fundamental engineering issues to be resolves, namely:
- the role of residual stresses;
- the influence of construction detail and stress concentrations;
- the use of material at temperatures well below the Charpy test temperature.
- It is important to leave the choice of Z quality testing to the fabricator except in very severe cases.
- It is important to specify a minimum mandatory level of inspection, including targeted NDT, after fabrication particularly for fatigue prone structures such as bridges.
- Safe life design should be sought for civil structures, in view of the potential high cost of in-service inspection.
6. Acknowledgements
The investigations and drafting work for the recent UK brittle fracture rules was carried out by TWI and UMIST on behalf of the UK Department of Transport. The assistance of P Lucha of UMIST and S Chakrabarti of the Highways Agency in this work is gratefully acknowledged.
7. References
- EN 10025: 'Hot rolled products of non-alloy structural steels. Technical delivery conditions'. European Committee for Standardization, Brussels.
- EN 10113: 'Hot rolled products in weldable fine grain structural steels - Part 1: General delivery conditions; Part 2: Delivery conditions for normalized/normalized rolled steels; Part 3: Delivery conditions for thermomechanical rolled steels'. European Committee for Standardization, Brussels.
- EN 10155: 'Structural steels with improved atmospheric corrosion resistance - technical delivery conditions'. European Committee for Standardization, Brussels.
- EN 10210-1: 'Hot finished structural hollow sections of non-alloy and fine grain structural steels - Part 1: Technical delivery requirements'. European Committee for Standardization, Brussels.
- EN 10219-1: 'Cold formed welded structural hollow sections of non-alloy and fine grain structural steels - Part 1: Technical delivery requirements'. European Committee for Standardization, Brussels.
- EN 10137: 'Plates and wide flats made of high yield strength structural steels in the quenched and tempered or precipitation hardened conditions - Part 1: General delivery conditions; Part 2: Delivery conditions for quenched and tempered steels; Part 3: Delivery conditions for precipitation hardened steels'. European Committee for Standardization, Brussels.
- BS 5400: 'Steel, concrete and composite bridges Part 3: Code of practice for the design of steel bridges, 2000; Part 6: Specification for materials and workmanship - steel, 1999; Part 10: Code of practice for fatigue, 1980 (1999)', British Standards Institution, London, UK.
- BS 5950: 'Structural use of steelwork in building - Part 1: Code of practice for design - rolled and welded sections, 2000; Part 2: 'Specification for materials, fabrication and erection - rolled and welded sections, 2001'. British Standards Institution, London, UK.
- ISO 10721: 'Steel Structures - Part 2: Fabrication and Erection'. International Organization for Standardization, Geneva, 1999.
- BS 5135: 'Process of arc welding carbon and carbon manganese steels'. British Standards Institution, London, UK, 1984.
- EN 1011: 'Recommendations for welding of metallic materials - Part 2: Arc welding of ferritic steels'. European Committee for Standardization, Brussels, 2001.
- ENV 1090: 'Execution of steel structures - Part 1: General rules and rules for buildings - Draft for Development'. European Committee for Standardization, Brussels, 1996.
- ENV 1993: 'Eurocode 3: Design of steel structures - Part 1 - General rules and rules for buildings - Draft for Development'. European Committee for Standardization, Brussels, 1992.
- ENV 1993: 'Eurocode 3: Design of steel structures - Part 2: Steel bridges Draft for Development'. European Committee for Standardization, Brussels, 1997.
- EN 1993: 'Eurocode 3: Design of steel structures - Part 1.10: Material toughness and through thickness properties - Final Draft'. European Committee for Standardization, Brussels, 2003. (To be published).
- EN 1993: 'Eurocode 3: Design of steel structures - Part 1.9: Fatigue strength of steel structures - Final Draft'. European Committee for Standardization, Brussels, 2003. (To be published).
- BS 153: 'Specification for steel girder bridges - Part 1: Materials, workmanship, protection against atmospheric corrosion'. British Standards Institution, London, UK, 1958 (1966).
- BS 499: 'Specification for the use of structural steel in building'. British Standards Institution, London, UK, 1959 (1966).
- PD 6493:1991: 'Guidance on methods for assessing the acceptability of flaws in fusion welded structures', British Standards Institution, London, UK, 1991.
- BS 7910:1999: 'Guide on methods for assessing the acceptability of flaws in fusion welded structures'. British Standards Institution, London, UK.
- EN 10164: 'Steel products with improved deformation properties perpendicular to the surface of the product - Technical delivery conditions'.
- Burdekin F M: 'Materials aspects of BS 5400: Part 6, Paper 4 in 'The Design of Steel Bridges', Ed. K C Rockey and H R Evans, Granada, ISBN 0 2461139 1, 1981.
- Wallin K: 'New improved methodology for selecting Charpy toughness criteria for thin high strength steels', IIW Document X-1290-94, 1994.
- Ogle M H: 'Weld quality specifications for steel and aluminium structures', Welding in the World/Le Soudage dans le Monde, 29(11/12), 1991, pp.341-362.
- Report of Royal Commission into the failure of the Kings Bridge, Melbourne, State of Victoria, Australia, 1963.
APPENDIX A
Procedure used for Calculation of Thickness Limits for Brittle Fracture Design Rules for Steel Bridges and Buildings in the UK
A.1 Introduction
The calculation procedure below was used for evaluating a range of design situations to determine general rules for selection of Charpy quality for steels used in bridges and buildings. This formed part of a programme of work carried out by the Structural Integrity Department at TWI, Cambridge, UK and the Civil Engineering Department at UMIST, Manchester, UK during 1995 and 1996. The results were used to derive the new bridges rules in BS 5400-3 (2002) and the new building rules in BS 5950-1 (2000).
The fracture mechanics assessment criteria followed the method laid down in BS PD 6493:1991 (now replaced by BS 7910:1999) which has been used as the basis of fitness-for-purpose assessment of welded joints in the process, power generation, offshore and structural industries since 1980. [19,20] At the same time account was taken of the developments taking place in the formulation of the Eurocode 3 brittle fracture rules and effort was made to provide a consistency between the UK and European developments.
A.2 Calculation procedure
A.2.1 Criterion and Stress Intensity Factor Calculation
A.2.1.1 Basic fracture criteria
The toughness of a structural element subjected to a given design tensile stress. The toughness of a structural element subjected to a given design tensile stress σ Ed was taken as sufficient if:
[1]
where K Ied is the applied stress intensity factor for the given design tensile stress σ Ed and assumed reference flaw size, K mat is the design fracture toughness at the minimum design temperature, γ m is the partial factor on fracture toughness to achieve the target reliability, ρ is the plasticity correction factor for the secondary stresses, K r is the permissible value of the fracture assessment diagram parameter at load ratio L r given by:
[1a]
[1b]
where σ net is the net section stress on the cracked section due to primary applied tensile stress, σ p and f y ( t) is the thickness dependent nominal yield strength. In view of the limited sizes of cracks compared to the gross cross section of a bridge member the net section stress were taken as equal to the applied stress in the region of a defect.
A.2.1.2 Stress intensity factor
The applied stress intensity factor was determined from the following:
[2]
where Y is the factor to allow for defect aspect ratio and position, and position, γ a is the partial factor on crack size and a d is the depth of the design crack imperfection (mm).
A.2.1.3 Applied stresses
For bridges the design tensile stress σ Ed was taken as:
[3]
where σ p is the primary tensile stress due to permanent actions and frequent variable actions, γ σ is the partial factor on applied stresses to achieve the target reliability, M K is the factor for weld toe stress concentration effects and σ s is the tensile value of the self-equilibrating secondary stresses such as residual stresses.
A.2.1.4 Residual stresses
For bridges the value of σ s for welding residual stresses relevant for defects contained within as-welded welds was taken as f y ( t), the thickness dependent nominal yield strength of the parent steel, but may be reduced depending on the loading parameter L r as follows:
[4]
For welded components which have been subject to a suitable post weld heat treatment to reduce residual stresses, the value of σ s was taken as 0.3 f y ( t).
A.2.1.5 Plasticity correction factor
The plasticity correction factor ρ was calculated from:
[5]
where 
A.2.1.6 Stress concentration factors
The value of Y.M K was derived from:
[6]
where p and q are constants for the particular weld geometry.
A.2.1.7 Partial factor on stress
The partial factor on best estimate of maximum applied stress γ σ was taken as [1].
A.2.1.8 Assumed crack dimensions
The design crack imperfection height was taken as follows:
[7]
The length should be 100mm and it should be surface breaking. These 'reference crack' dimensions have been chosen to allow for the possibility of limited fatigue crack growth at welded details in service. For these conditions to be appropriate it is essential that all weld toe geometry regions lying in fatigue sensitive parts of the bridge should be subject to post-fabrication non destructive testing and demonstrated to be free from defects.
A.2.1.9 Partial factor on crack depth
The partial factor on best estimate of crack depth γ a was taken as 1.
A.2.1.10 Influence of thickness on yield strength
The thickness dependent nominal yield strength f y ( t), (N/mm 2 ) was determined from:
[8]
where t is the element thickness and t 0 is a reference value of t equal to 1mm.
A.2.2 Derivation of Design Fracture Toughness
A.2.2.1 Use of Charpy impact test data
The fracture toughness was correlated with the mean of three Charpy V notch impact test results as follows:
[9]
where T min is the minimum design temperature, T 27J is the temperature for 27 Joules energy absorption in the Charpy test for the grade of steel concerned (average of three test results), b eff is the effective thickness of the material concerned at the welded joints and T k represents the effect of the standard deviation in the correlation between K mat and T 27J for a required probability level given by K. Wallin [23] as:
[10]
The value of K mat from Eqn.[9] is an estimate of the fracture toughness in MPa √ m with a probability of P f of not being exceeded at a temperature T min . It should be noted that the temperature T 27J is the temperature for the average of Charpy test values but if this is a minimum specified value it will represent the minimum average for an expected distribution.
A.2.2.2 By fracture mechanics tests
Alternatively the design fracture toughness K mat may be determined by taking the minimum of three valid standard fracture toughness tests for K Ic or equivalent at the minimum design temperature.
A.2.2.3 Partial factor on fracture toughness
The partial factor on characteristic value of fracture toughness γ m was taken as 1.
A.2.3 Design Temperature
A.2.3.1 Derivation of minimum steel temperature
The value of the design temperature T min for the steel at the point of potential fracture initiation in an external structure exposed to the weather should be determined from the minimum air temperature at which the design stress σ Ed applies together including any allowance for thermal radiation effects considered necessary.
A.2.3.2 Return period for minimum temperature
The value of T min should be taken as the value with a return period of 100 years.
A.3. References
(See Section 7 of the main paper).