Austenite and ferrite
Austenite was originally used to describe an iron-carbon alloy, in which the iron was in the face-centred-cubic (gamma-iron) form. It is now a term used for all iron alloys with a basis of gamma-iron. Austenite in iron-carbon alloys is generally only evident above 723°C, and below 1500°C, depending on carbon content. However, it can be retained to room temperature by alloy additions such as nickel or manganese. Similarly, ferrite was a term originally used for iron-carbon alloys, in which the iron was in the body-centred cubic (alpha- or delta-iron) morphology, but is now used for the constituent in iron alloys, which contains iron in the alpha- or delta-iron form. Alpha ferrite forms by the slow cooling of austenite, with the associated rejection of carbon by diffusion. This can begin within a temperature range of 900°C to 723°C, and alpha-ferrite is evident to room temperature. Delta ferrite is the high temperature form of iron, formed on cooling low carbon concentrations in iron-carbon alloys from the liquid state before transforming to austenite. In highly alloyed steels, delta ferrite can be retained to room temperature.
When iron carbon alloys transform from austenite on cooling, the solubility limit of carbon in ferrite is commonly exceeded. Under slow cooling conditions, carbides are formed, and at faster cooling rates carbon may be trapped in solid solution.
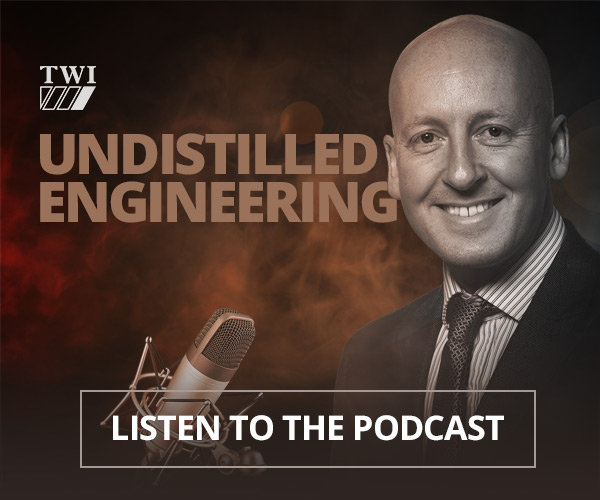
Pearlite
Pearlite is usually formed during the slow cooling of iron alloys, and can begin at a temperature of 1150°C to 723°C, depending on the composition of the alloy. It is usually a lamellar (alternate plate) combination of ferrite and cementite (Fe3C). It is formed by eutectoid decomposition of austenite upon cooling by diffusion of C atoms, when ferrite and cementite grow contiguously, C precipitating as Fe3C between laths of ferrite at the advancing interface, leaving parallel laths of Fe and Fe3C which is pearlite.
Martensite
Martensite is formed in steels when the cooling rate from austenite is sufficiently fast. It is a very hard constituent, due to the carbon which is trapped in solid solution. Unlike decomposition to ferrite and pearlite, the transformation to martensite does not involve atom diffusion, but rather occurs by a sudden diffusionless shear process. The term is not limited to steels, but can be applied to any constituent formed by a shear process which does not involve atom diffusion or composition change. The martensite transformation normally occurs in a temperature range that can be defined precisely for a given steel. The transformation begins at a martensite start temperature (Ms), and continues during further cooling until the martensite finish temperature (Mf) is reached. Ms can occur over a wide range, from 500°C to below room temperature, depending on the hardenability of the steel. The range Ms to Mf is typically of the order of 150°C. Many formulae have been proposed to predict the martensite start temperature. Most are based on the composition of the steel, and a selection are listed in the following table:
Proposer's name | Formula |
Savage (1942) |
Ms (°C) = 500 - 300C - 35Mn - 20Cr - 15Ni - 10Si - 10Mo |
Grange and Steward (1946) |
Ms (°C) = 538 - 341C - 39Mn - 39Cr - 19Ni - 28Mo |
Steven and Haynes (1956) |
Ms (°C) = 561 - 474C - 33Mn - 17Cr - 17Ni - 21Mo |
Andrews I (1965) |
Ms (°C) = 539 - 423C - 30.4Mn - 12.1Cr - 17.7Ni - 7.5Mo |
Andrews II (1965) |
Ms (°C) = 512 - 453C +217C2 - 16.9Ni +15Cr - 9.5Mo - 71.5CMn - 67.7CCr |
Beres and Beres (1993) |
For 0.03<C<0.35,
Ms (°C) = 454 - 210C + 4.2/C - XNi -YMn - ZCr(eq) - 21Cu
Where X, Y, Z are factors that change with the appropriate elemental composition
Cr(eq) = Cr + Mo + 1.5Si + W + V + Al
|
Beres and Beres [1] stated that their formulae were within 40°C of the actual Ms , in all cases studied, whereas other formulae had larger scatter bands. More recently, Ms models have been developed through the use of neural networks, trained on experimental data and using further data to validate and test the model, a reasonable approximation of Ms can be identified. Such models are available on the web [2] and can be used with compositional information. Neural networks based on the relationship between the chemical composition, transformation temperature and kinetics during continuous cooling enable calculation of a CCT diagram for the steel. These also take into account the influence of alloying elements on the phase transformation curves, as well as the resulting hardness. It is also possible to predict quantitatively the microstructure of the steel e.g. the percentage of ferrite, pearlite and bainite etc. [3]
Models combining the kinetics of martensitic transformation with mechanics, in view of microstructural development are also applicable. Finite element analysis enables evaluation of the local stress and strain fields as well as monitoring the kinetics of martensitic transformation and development of the understanding on critical parameters such as effect of austenite grain size on the resulting martensitic microstructure. [4]
In-situ experimental studies based on synchrotron radiation can also result in valuable data to support computer models, as real-time study of such diffusionless phase transformations will be crucial to broaden the understanding of microstructural development and related structure-property relationships. [5]
Bainite
Bainite is formed at cooling rates slower than that for martensite formation and faster than that for ferrite and pearlite formation. There are two forms of bainite, known as upper and lower bainite.
Upper bainite generally forms at temperatures between 550 and 400°C. There are several proposed formation mechanisms, based on the carbon content and transformation temperature of the steel, resulting in slightly different morphologies. Low carbon steels exhibit fine bainitic laths, nucleated by a shear mechanism at the austenite grain boundaries. Carbon solubility in bainitic ferrite is much lower than in austenite, so carbon is rejected into the austenite surrounding the bainitic ferrite laths. When the carbon concentration in the austenite is high enough, cementite nucleates as discrete particles or discontinuous stringers at the ferrite/austenite interfaces. As the carbon content increases, the cementite filaments become more continuous, and at high carbon contents, the bainitic ferrite laths are finer with the cementite stringers more numerous and more continuous. The structure can appear more like pearlite, and is termed 'feathery' bainite.
Lower bainite generally forms at temperatures between 400 and 250°C, although the precise changeover temperature between upper and lower bainite depends on the carbon content of the steel. The transformation nucleates, like upper bainite, by partial shear. The lower temperature of this transformation does not allow the diffusion of carbon to occur so readily, so iron carbides are formed at approximately 50-60° to the longitudinal axis of the main lath, contiguously with the bainitic ferrite. With low levels of carbon, the carbide may precipitate as discrete particles, following the path of the ferrite/austenite interface. However, the overall mechanism of lower bainite formation is independent of carbon content in the main. The appearance of lower bainite strongly resembles that of martensite, but lower bainite is formed by a mixture of shear and diffusional processes rather than just shear.
References
- Beres L and Beres Z: 'Neue Beziehung zur Bestimmung der Martensitbildungstemperatur der Stahle', Schweisstechnik (Wien), 47 (12), December 1993, pp186-188
- Sourmail T and Garcia-Mateo C: 'A model for predicting the Ms temperatures of steels' Computational Materials Science Volume 34, Issue 2, September 2005. pp213-218.
- L A Dobrzanski & J Trzaska: 'Application of neural networks to forecasting the CCT diagrams', Journal of Materials Processing Technology, Vol. 157-158, 2004, pp 107-113.
- G Reisner, E A Werner and F D Fischer: 'Micromechanical modelling of martensitic transformation in random microstructures', Int. Journal of Solids & Structures, Vol. 35, Issue 19, 1998, pp. 2457-2473.
- R G Thiessen et al: 'Phase field modelling and synchrotron validation of phase transformations in martensitic dual phase steel', Acta Materialia, Vol.55, Issue 2, 2007, pp 601-614.